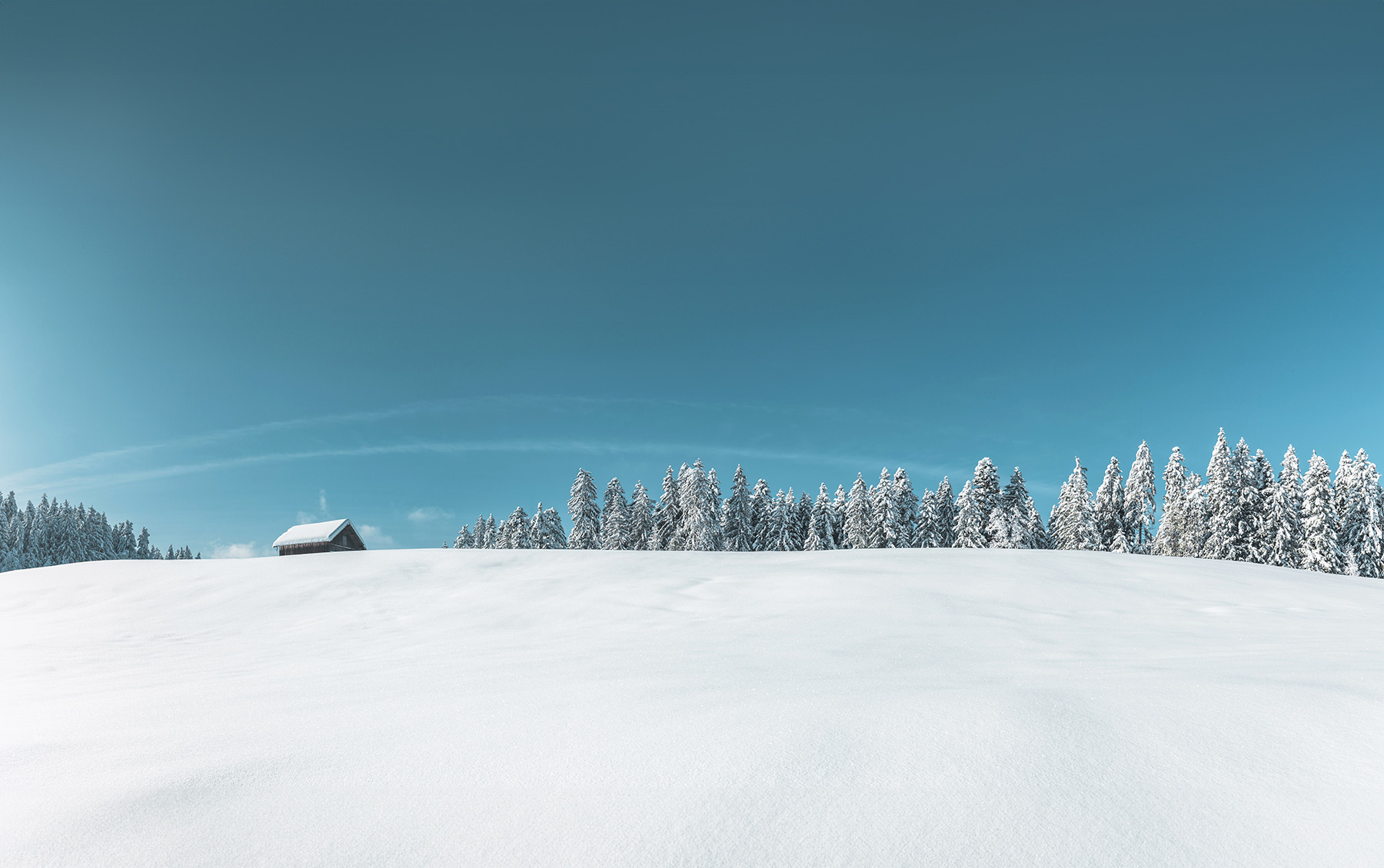
By Robert L. Reid
Underground work in cold regions can feel familiar at times, and at other times it may seem unique, even strange. From climate change and the impermanence of permafrost to the varying conditions under which ground must either be thawed or frozen, the subterranean world of cold regions is always challenging for civil engineers.
The design and construction of underground engineering projects in the Northern Hemisphere’s cold regions can sometimes seem counterintuitive, even surreal. This is a world of snow and ice in which too much of that snow can cause frozen sites to warm up, frozen ground might need to be deliberately thawed, or a thawed site might need to be refrozen.
Here, the ironically named permafrost can be anything but permanent, and a normally desirable southern exposure can create challenges. In this environment, soil conditions listed as “poor” in certain ways might be good to work in, while conditions labeled as “rich” can pose problems. And often, the engineers and contractors working in these cold regions must operate within an especially short construction window that forces them to wait for a change in the season before completing certain tasks.
This article focuses on underground engineering projects in the Northern Hemisphere’s cold regions because the extensive land masses there offer considerably more opportunities for such work than the Southern Hemisphere’s cold regions.
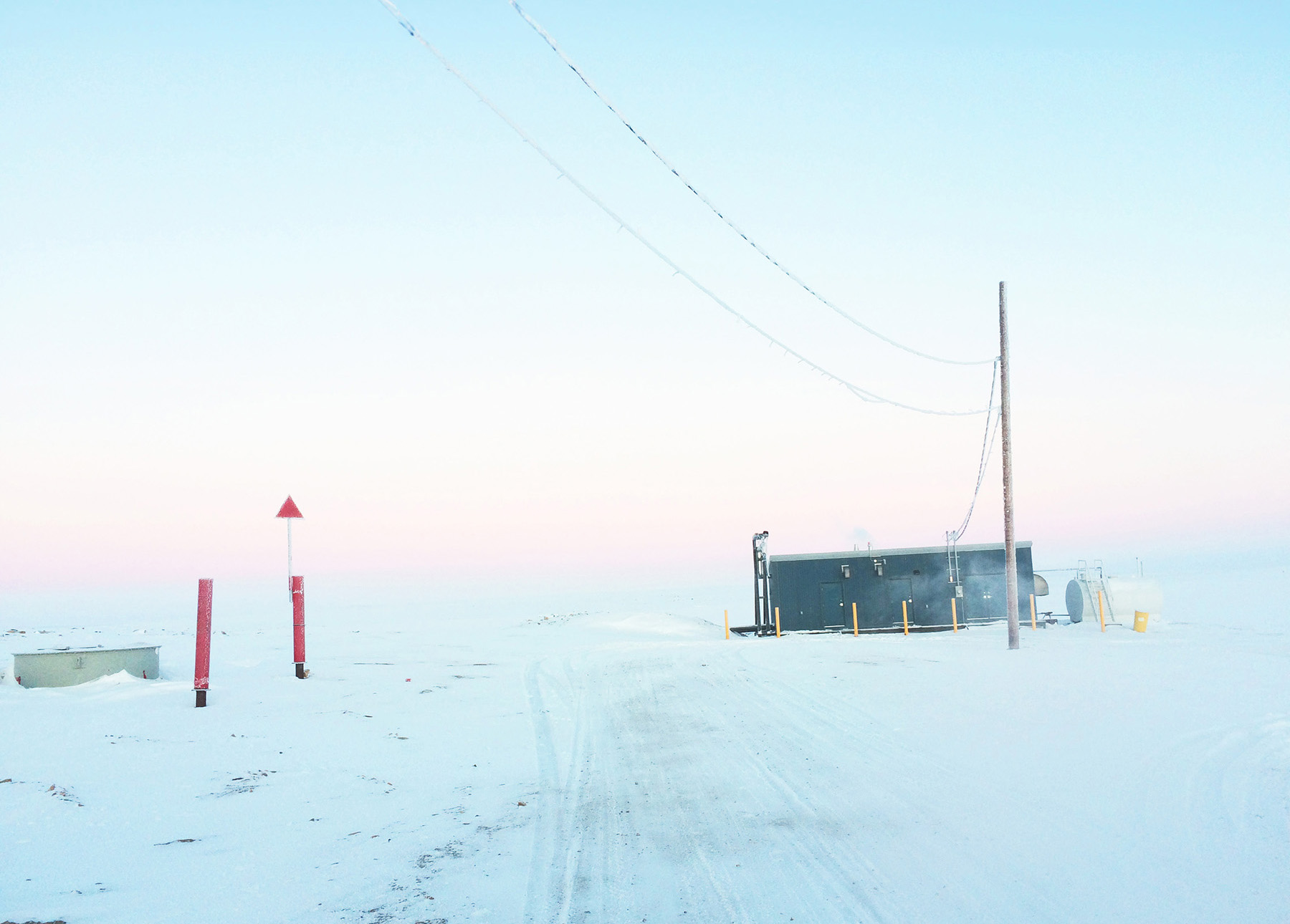
The challenges of underground engineering in northern climes are highlighted by the diversity of the region’s subsurface conditions. For example, the farther north a project in Alaska or Canada might be, the deeper the Arctic cold will penetrate the ground. On the Alaska North Slope, almost all the ground is permafrost — soil that has remained frozen for at least two years. It is distinguished from seasonally frozen ground in more southern regions like North Dakota or Wisconsin, explains Kevin Bjella, P.E., research Arctic civil engineer at the Cold Regions Research and Engineering Laboratory in Fairbanks, Alaska.
The lab is part of the U.S. Army Corps of Engineers’ Engineer Research and Development Center. The permafrost in Northern Alaska is so pervasive that it is called continuous permafrost. It is extremely cold — just 12 F in some areas — and can be more than 1,000 ft thick.
Moving southward, however, permafrost grows warmer and thinner. In central Alaska, around Fairbanks, the permafrost’s temperature can approach the freezing point (32 F) while its thickness might measure only 100 to 200 ft, Bjella says. Moreover, the permafrost in central Alaska is considered discontinuous, which means that an area of permafrost can be adjacent to an area of thawed ground, which is near another area of permafrost, then more thawed ground, and so on, Bjella explains.
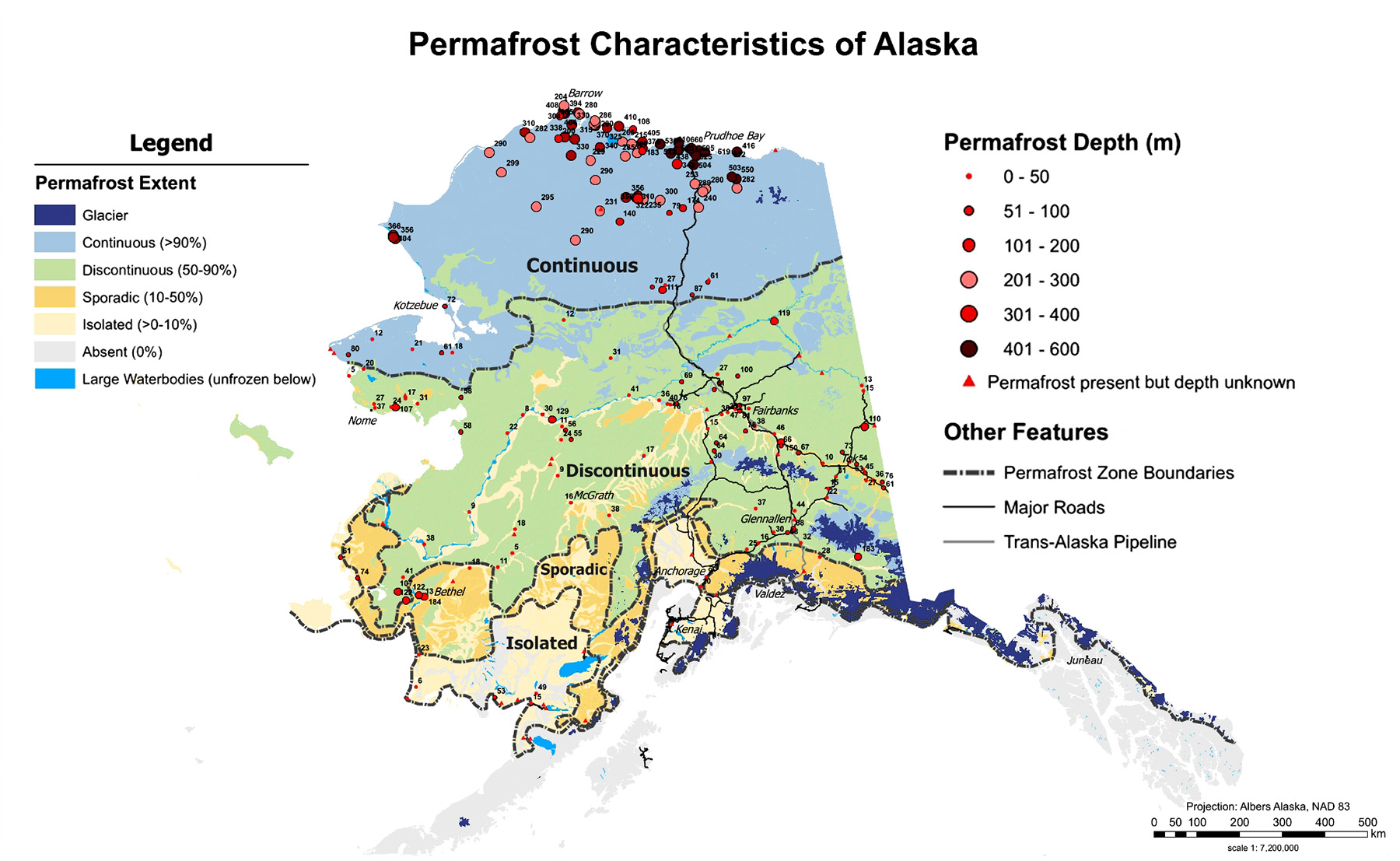
Several factors cause this discontinuity of permafrost, Bjella notes, including warmer air temperatures — especially now, as climate change accelerates — and geographic orientation. Southern-facing hills around Fairbanks will generally have less permafrost than the northern sides of the hills because of solar exposure. Areas with extensive vegetation will have more permafrost than areas heavily covered in snow because the plants shade the permafrost and keep it cold, while snow can insulate the ground and keep it warm, preventing annual refreezing.
In southern Alaska, near Anchorage, the permafrost is even less prevalent and is considered isolated or sporadic, Bjella adds.
Tough but sensitive
Under the coldest conditions, permafrost is an extremely tough material to excavate or drill into, which is why few projects constructed on permafrost include basements or other underground levels, Bjella says. At the University of Alaska Fairbanks, where Bjella earned his bachelor’s and master’s degrees in geological and Arctic engineering, the extreme challenges of working in permafrost are on public display: An exhibit in the engineering school building houses a pair of foundation piles from a construction project in the 1970s that were supposed to be driven into the ground.
Instead, the piles “compressed like an accordion,” Bjella says. The construction crew apparently “had no idea the piles weren’t actually going down into the ground, that they were literally just squeezing together,” he explains. So, instead of being part of an Arctic structure’s foundations, the piles serve “as a dramatic demonstration of how stiff (permafrost) ground can be.”
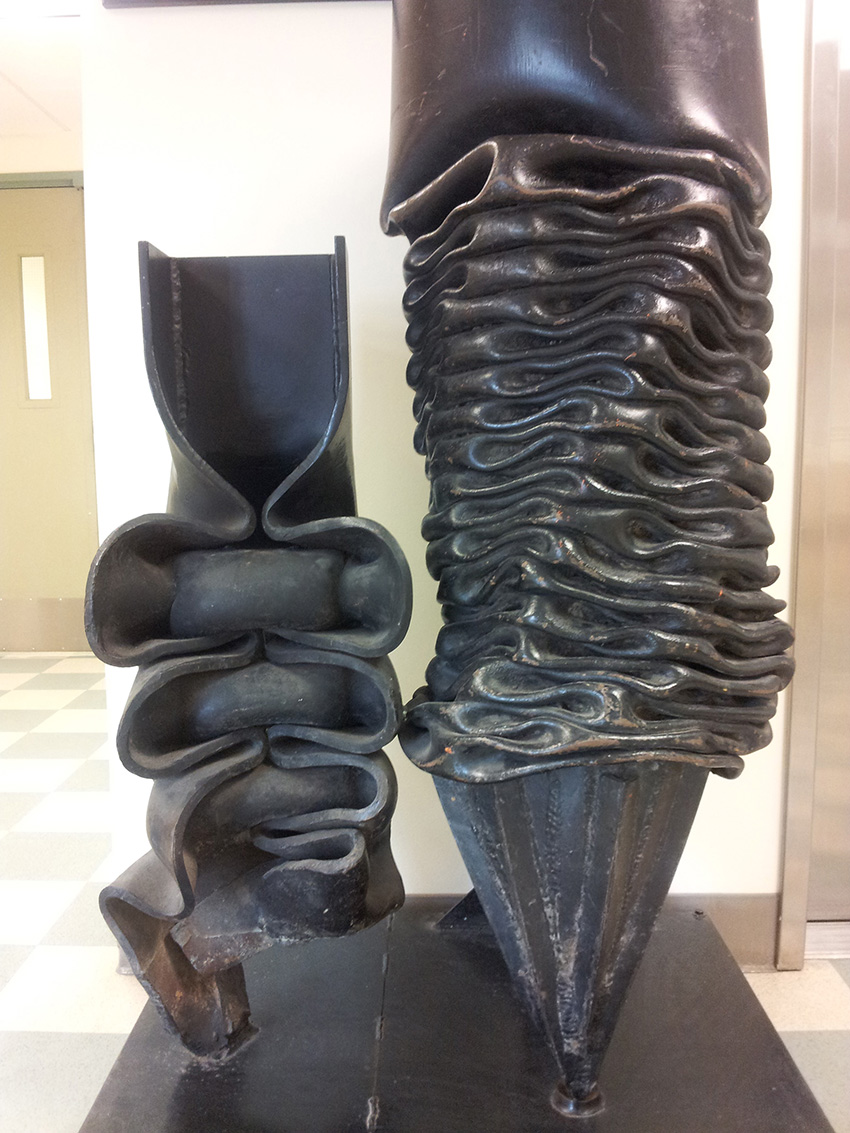
At the same time, permafrost can be extremely sensitive to changes in temperature, especially when the permafrost is in a discontinuous area that may be only a few tenths of a degree below freezing, notes Wendy Presler, P.E., M.ASCE, a senior associate and geotechnical engineer in the Fairbanks office of Shannon & Wilson.
“It only takes a degree or two of temperature change to have a dramatic effect on permafrost,” Presler explains, “and as that happens, as the environment warms, our winters aren’t as cold and the summers are warmer and longer, so the permafrost temperatures are rising.”
Fifty years ago or so, buildings in Canada’s Yukon territory often used permafrost as their foundation system — sort of like a frozen slab on grade. But today, many of those buildings are sinking into the ground because the permafrost is thawing.
Essentially, this means engineers are becoming unable to rely on permafrost for structural stability, says Arlen Foster, PEng, Stantec’s infrastructure practice lead for Northern Canada.
Moreover, the structures built into or atop continuous or discontinuous areas of permafrost — from buildings to roads to tunnels — can themselves impart heat into the underlying soil. So too can the structural elements such as foundation piles and buried concrete utility tunnels — often dubbed “utilidors,” says Presler — that are set in the frozen ground. All these heat sources represent potential challenges for civil engineers working in cold regions.
Sometimes the solution to such underground challenges is to limit what is put in the ground in the first place. In Canada’s Nunavut territory, buildings are elevated on piles because “you want the cold wind to flow under the building so the ground temperature and the subsurface temperature aren’t impacted by heat from the structure,” says Matthew Follett, PEng, an associate and civil engineering lead for Stantec in Nunavut. The infrastructure for water and wastewater systems are often elevated as well to protect the ground from potential heat sinks that degrade the permafrost, says Foster.
Stable and unstable
The specific composition of the permafrost also matters, since some permafrost includes large quantities of ice — dubbed “ice-rich” — while other areas of permafrost are considered “ice-poor.” If ice-rich permafrost thaws, it is considered “thaw unstable” because the more ice that is in the ground, the less bearing capacity the soil will have if the ice melts, Bjella says. That puts structures built on ice-rich permafrost at risk of dangerous settlement if the underlying soils thaw.
Many houses and cabins in the ice-rich hills north of Fairbanks sit on piles driven into the permafrost, Bjella says. Because Fairbanks experiences longer and warmer summers than other parts of the state, the foundations of these structures can weaken due to heat transmitting down through the piles. “It is very common to find structures settling on the south side, listing on the south side, due to the heat conduction,” he notes.
By contrast, the building in which Bjella works at Fort Wainwright in Fairbanks was constructed in the 1950s on ice-poor ground, which is considered thaw stable because it was composed of mostly frozen gravel. So, when that soil thawed, it retained its bearing capacity. The structure even has a heated basement, but “we’ve had no problems because it was thaw stable,” he says.
Alaskan ground conditions can be so variable that the state’s department of transportation will create several design profiles for each project. If the ground is thawed and gravelly, the Alaska DOT will have a cross section for the contractor to follow for that specific type of soil, Bjella says. “Or if it’s frozen gravel, they’ll have a second profile to tell the contractor that if you encounter these conditions, this is what you will build, which might involve adding a little more gravel or height to the embankment or adding some geotextiles as insulation,” Bjella notes. “And then if they get into ice-rich, thaw unstable permafrost, then there’ll be a third profile for that.”
Ground exploration
When working in or around permafrost, the goal is generally — but not always — quite simple: “If it’s frozen, keep it frozen,” says John Thornley, Ph.D., P.E., BC.GE, M.ASCE, a vice president and senior geotechnical engineer in WSP USA’s Anchorage office. To that end, “temperature is everything in permafrost design,” he explains, so engineers often rely on sensors called thermistors to measure underground temperatures in Arctic regions and monitor how those temperatures change seasonally.
In many cases, permafrost can be identified through aerial imagery, Thornley adds. “If you look at (the) North Slope, you’ll see all these sorts of polygonal shapes, which are a distinct feature of permafrost,” he says. The polygonal forms are created through a combination of soil contraction and the freezing and expansion of water above the permafrost.
Satellites can also help monitor Arctic permafrost, as was discussed during a May 15 webinar, “Big Imagery in Action: Auto Detection of Arctic Permafrost,” presented by ASCE’s Utility Engineering & Surveying Institute. The webinar featured the National Science Foundation-funded research of Chandi Witharana, Ph.D., an assistant professor of remote sensing in the Department of Natural Resources and the Environment at the University of Connecticut, who is using supercomputers and artificial intelligence tools to analyze millions of images of Arctic permafrost taken from space.
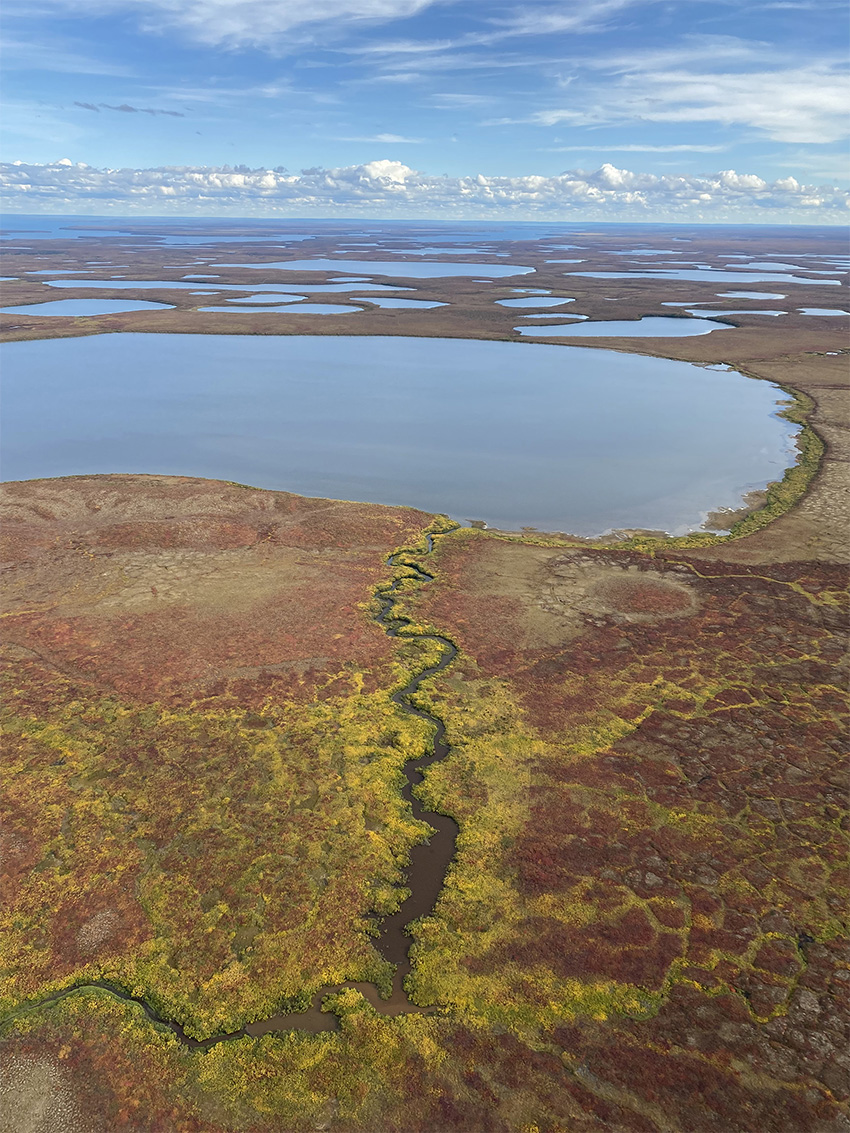
Following the collapse of a roadway in Alaska because of thawing permafrost, Stantec also used satellite images, as well as data from lidar and other technologies, to measure “subsidence in permafrost areas at local and regional levels to predict ground deformation caused by permafrost thaw,” and also to determine the types of slopes most susceptible to such damage, according to an article on the company’s website, “Satellite Imagery Predicts Infrastructure Damage from Permafrost Thaw.”
Because the stability or instability of thawed ground in cold regions can differ dramatically from one location to another — at times, changing over distances of less than a dozen feet, according to Bjella — engineers often drill bore holes to take physical samples of the geotechnical conditions.
But drilling is time-consuming and expensive, he adds, so in recent years engineers have also relied on such techniques as ground-penetrating radar and surface-based geophysics. Geophysical methods include electrical resistivity, which can distinguish ice-rich from ice-poor ground and even determine if the ground has thawed. “It draws a fairly decent, accurate picture, especially if you’re experienced using it,” Bjella says.
Extensive geotechnical exploration and monitoring, as well as 2D and 3D modeling of the underground conditions, can also help identify if liquid water is present underground, which is possible even in permafrost, and “to determine what sort of ground freezing or refrigeration we need to implement,” says Thornley.
Although the ground-freezing techniques on these projects are similar to those in urban excavations far south of the Arctic, Thornley acknowledges the irony of having to freeze the ground in cold regions. The ground around portals for highway or railroad tunnels, for instance, as well as pipes that are sunk into permafrost to extract hot oil or gas, can also require ground-freezing measures because otherwise the thawing soils might make the site unstable, dragging down the pipe casing, portal structure, or other infrastructure, Thornley says.
Seismic concerns
Alaska is a highly seismic region, which — combined with climate change — is especially challenging to engineers, because when permafrost thaws, the remaining soil can be loose sands and gravels. Although those materials had been “locked up” in the permafrost, Thornley notes, after thawing, the soil becomes subject to liquefaction. This was demonstrated dramatically during the 7.9 magnitude 2002 Denali Fault earthquake. The Denali quake was the “strongest ever recorded in the interior of Alaska” and spread liquefaction through much of the Tanana Valley, according to the United States Geological Survey’s fact sheet on the event, “Rupture in South-Central Alaska — The Denali Fault Earthquake of 2002.”
Fortunately, the Denali quake struck in a sparsely populated region, resulting in limited structural damage and no fatalities, the USGS noted. But it was a dramatic wake-up call for engineers in Alaska, who had not previously thought the ground conditions in the Fairbanks region were liquefiable, says Presler.
The discovery “changed the way we look at projects,” Presler notes. For example, at the Fairbanks Memorial Hospital, which had been constructed prior to the Denali quake, the ground beneath the site of a planned new surgery center was improved through compaction grouting and vibro compaction methods to help prevent liquefaction during future seismic events.
There were similar concerns for Eielson Air Force Base, also near Fairbanks, during a project to prepare the base for the new F-35 aircraft. The work, involving the Corps, WSP, and Shannon & Wilson, among other firms, included a refurbished runway, hangars, and other structures. These facilities were planned for ground that was previously developed as well as ground that had never been developed.
As such, the geotechnical conditions varied from thawed soils to untouched permafrost. When ground surveys indicated a risk of liquefaction beneath the proposed runway and possibly in other areas, the Air Force decided that the general rule of keeping frozen ground frozen did not apply. Instead, they deliberately thawed the entire site, says Thornley.
To do so, they spread heat blankets across the surface and inserted electric heating probes into the ground, says Presler. Thawing generally started in the most upgradient area of the site, and the blankets and probes were periodically moved to the next area to be thawed, following the direction of groundwater flow. This work thawed approximately the upper 40 ft of a roughly 40,000 sq ft area. Then, because thawed soils in that area were known to be fairly loose, dynamic deep compaction was used to improve the site conditions for construction, Presler says.
Because the thawing process alone took three months to complete, most of the construction season was used up just preparing the site for the foundations of the new facilities. The rest of the work had to wait “until the following spring before they could finally start building,” Presler says.
Pipeline protection
One of the most successful examples of engineering in the Northern Hemisphere’s cold regions — as well as an exemplar of the underground challenges that confront engineers there — is the Trans-Alaska Pipeline System. Designed and constructed in the 1970s by Alyeska Pipeline Service Co., and still operated and maintained by that company, the 800 mi long conveyance from the Prudhoe Bay oil fields in northern Alaska to the southern Alaskan port of Valdez was originally envisioned as a buried pipeline.
However, that design was impractical for numerous reasons, Bjella explains, most notably because the oil is transported through the pipeline starting at about 140 F when pumped from the ground. So even with insulation around the pipes, the heat would have been transmitted into the permafrost, causing catastrophic thawing, he says.
Roughly half the pipeline did end up underground — in bedrock, in already thawed ground, and even in some areas of questionable soil where the route had to pass under highways or animal crossings or to avoid potential landslide or avalanche areas, according to Alyeska’s website. The other half, however, is elevated on a piled vertical support system that features both passive and active cooling technology designed to keep the permafrost frozen around the piles.
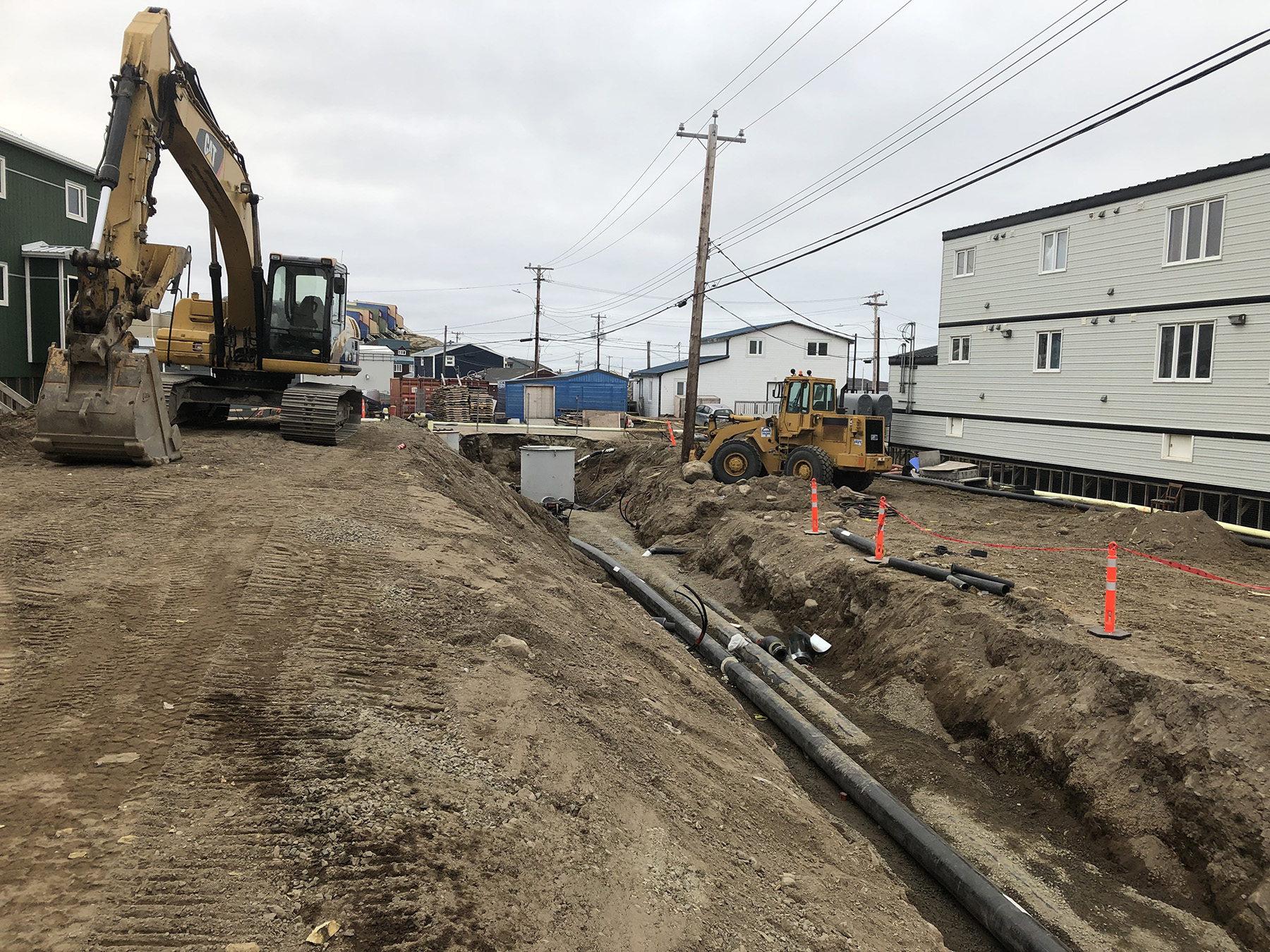
The passive cooling technology uses nearly 100,000 devices called thermosyphons that were originally developed by a Corps engineer. This system uses sealed metal tubes sunk into the permafrost that extend aboveground. The tubes are filled with a gas slightly under pressure — originally ammonia but now generally carbon dioxide, Bjella says — that absorbs the heat that would otherwise thaw the permafrost and releases that heat to the atmosphere through radiating fins at the aboveground part of the tubes.
An active cooling system is also used at certain locations along the pipeline, relying on refrigerated brine circulated through underground pipes that absorb the heat from the support structures, according to the Public Broadcasting Service website article “Pipeline Designs to Protect Permafrost.” The article is an online companion to a PBS documentary, The Alaska Pipeline, which aired in April 2006. The refrigerated brine system is powered by electric motors, found in nearby buildings, that also feature heat exchangers to remove the heat from the brine and send it to the outside air.
During the 2002 Denali earthquake, the pipeline experienced violent shaking and was slightly damaged, but it did not break. This was, in part, because of “careful engineering to meet stringent earthquake design specifications based on geologic studies,” according to the USGS.
(Climate)-changing designs
As the climate warms, engineers are designing foundations for Arctic structures with an assumption that what is permafrost today might not be permanently frozen in the future. For Presler, this means designing deep piles that will still function even if the entire depth of soil along the length of the pile thaws. If permafrost thaws and the water drains away, the remaining soil might move downward along the pile, generating a downward drag that must be considered when calculating the pile’s capacity, Presler says.
On the archipelago of Svalbard, roughly halfway between the Norwegian mainland and the North Pole, the government of Norway has built a remarkable Arctic structure deep into a permafrost mountain. Completed in 2008, the Svalbard Global Seed Vault houses more than 4.5 million seed samples as a hedge against climate change or some other event that could lead to agricultural apocalypse. The vault extends more than 100 m into the mountain, in which three chambers and an artificial cooling system safeguard the seed samples at temperatures as low as minus 18 C.
The seed vault is managed jointly by the Norwegian Ministry of Agriculture and Food, the Nordic Genetic Resource Center (also known as NordGen), and the Crop Trust. The site originally featured a concrete entrance portal and a steel-framed tunnel leading to the rock chambers. But in 2016, thawing permafrost at the entrance resulted in unexpected water intrusion in the tunnel. Although the seeds were reportedly never in danger, Statsbygg, the Norwegian government’s entity for planning, constructing, and managing public buildings, was tasked with renovating the facility.
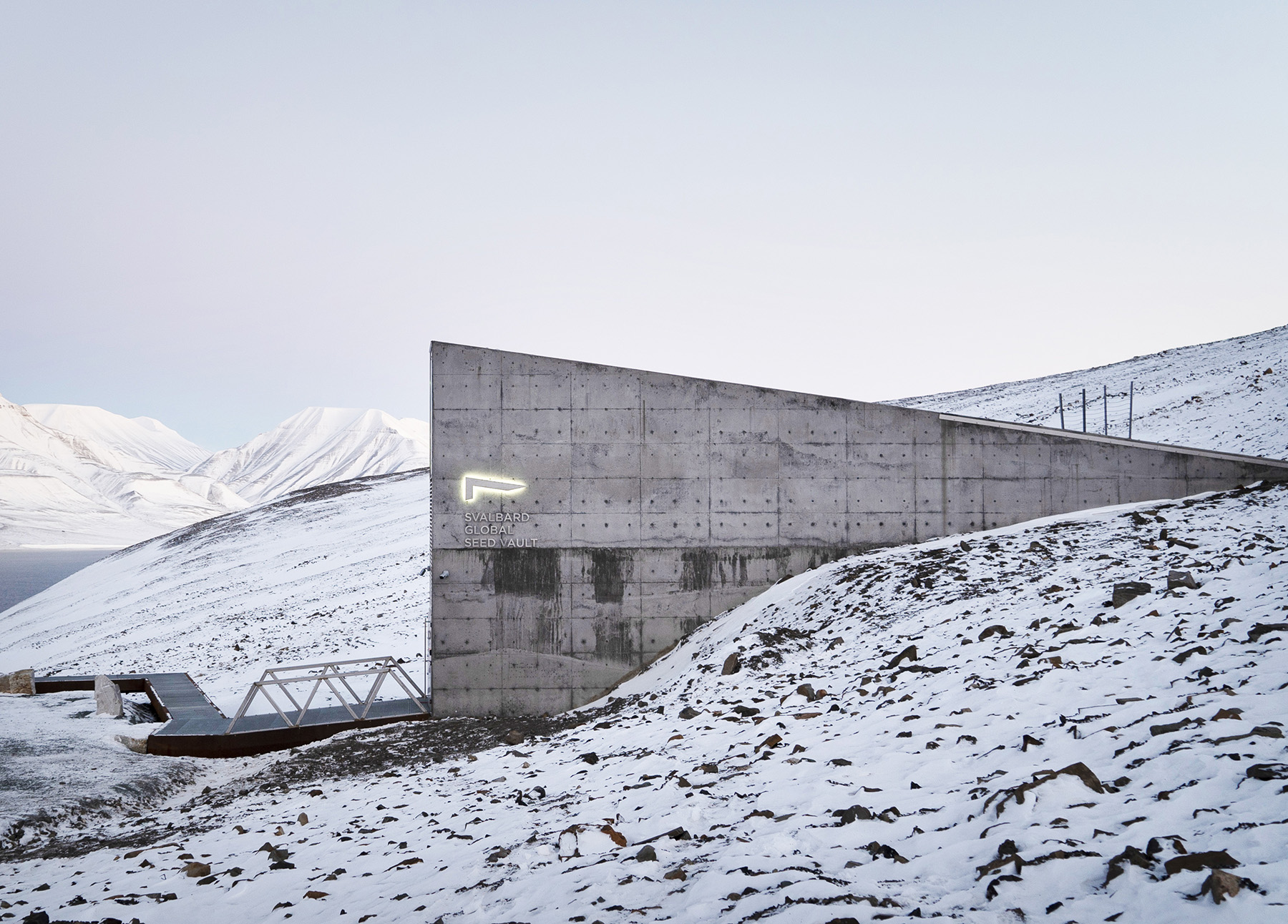
The changes included replacing the steel tunnel with a prestressed concrete structure that is kept constantly under pressure to make it watertight, says Knut-Ola Lunde, a Statsbygg civil engineer.
In addition, the soil around the tunnel was put back in place in layers with tubes that circulate a frigid, salty water designed to freeze any external water that trickles down toward the tunnel. “We artificially remade the permafrost above the tunnel,” Lunde explains.
The renovations also relocated potential heat sources — for the lights, the cooling system, and other amenities — from inside the mountain to a new service building outside the tunnel aboveground.
Designed by the architecture firm Snøhetta, the service building was completed in 2019. The structure is supported “on heavy steel (piles) ... on a solid foundation mounted to the ground to ensure it doesn’t get disrupted by the permafrost,” according to Snøhetta’s website.
Each spring, the first 2 m of permafrost thaws, “creating forces similar to a powerful river, and the (piles) ensure that the pressure from these forces keeps the building steady and secure.”
Teaching tunnel
For civil engineers who want to learn more about permafrost and other aspects of the Arctic underground, there might be no better teaching tool than a tunnel dug through the frozen ground of Fox, Alaska. Originally constructed during the Cold War as a military facility, the Permafrost Tunnel Research Facility — part of the Corps’ Cold Regions Research and Engineering Laboratory — now provides engineers and scientists a unique setting “to study warm, ice-rich, fine-grained permafrost in situ — allowing time for detailed research and sampling,” according to a Corps fact sheet on the tunnel.
The tunnel originally measured just 500 ft long but has since been expanded to approximately 1,850 linear ft of underground passages, says Bjella, who was the project engineer and managed all phases of construction during the expansion. In addition to various underground ice formations, such as vertical ice wedges and horizontal ice lenses, the tunnel also offers researchers access to “45,000 years of details” about the soil, the fact sheet notes.
This includes bacteria and organics, from plants to mammoth bones. According to the fact sheet, “The tunnel’s walls reveal how a sequence of climate shifts have affected the permafrost in central Alaska during and following the last Ice Age, enabling researchers to better predict future climate change effects on permafrost.”
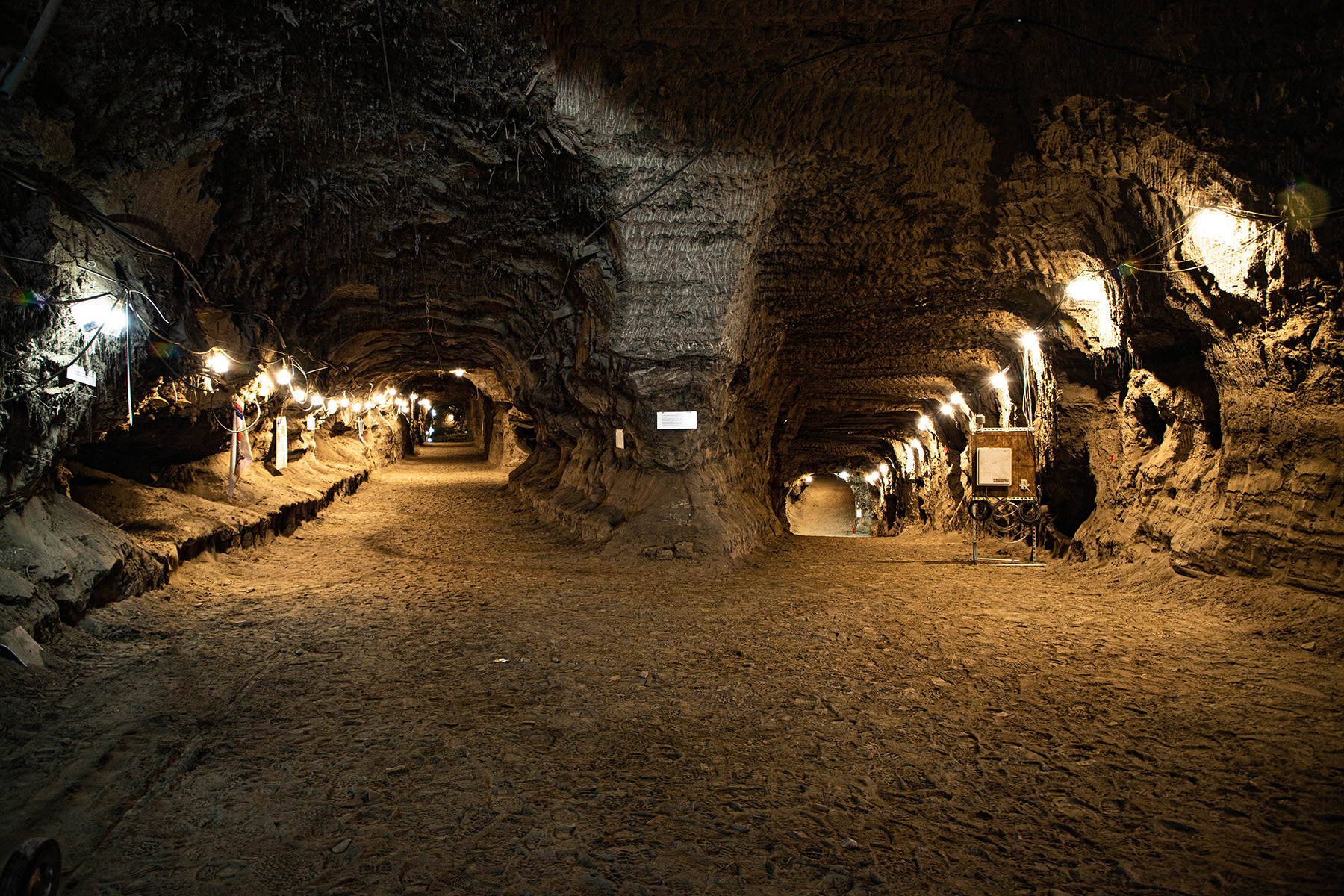
Construction in the tunnel and ongoing maintenance work also provide engineers with insights into the challenges of underground engineering in permafrost. Bjella says the construction crew expanded the tunnel with a medium-sized excavator fitted with a cutting head powered by an internal combustion engine.
Electrically powered mining equipment would have worked better, but it was not available. So, the excavation posed extra challenges related to exhaust and air quality issues, as well as the heat caused by the machine.
Excavation work could be done only in winter months to avoid thawing the permafrost, because that was when “we could get really cold air down into the tunnel and deliver it to the excavation face,” Bjella explains. Moreover, due to the presence of discontinuous permafrost in the region, the construction crew worried about digging into thawed areas in which the organic material might have decomposed, forming gas pockets filled with explosive methane.
Underground water in thawed areas presented another potential threat, so the main excavation machinery was preceded by a small, 4 in. diameter horizontal drill. This way, if the drill encountered dangerous methane gas or water, the crew could plug the hole quickly before inundating the tunnel, Bjella says.
To help mitigate those concerns, the tunnel is kept at a constant temperature of minus 5 C year-round, Bjella says. In the summer, the tunnel is kept cold through mechanical cooling; in the winter, “we open up the doors” and a powerful 150 hp fan pulls in the frigid air. These measures help prevent the tunnel roof from moving downward under its own weight and keep the walls from moving inward under the lateral pressure, Bjella says.
Occasionally, Bjella adds, the artificial cooling has failed temporarily during the summer, and the results were readily apparent. As the temperatures inside the tunnel warmed up to near the freezing point, “we’ve seen the tunnel very slowly start to close up,” he says. “It’s only millimeters of movement per week, but if it were left that way over time, it would close up on itself and collapse.”
Robert L. Reid is senior editor and features manager of Civil Engineering.
This article first appeared in the September/October 2024 issue of Civil Engineering as “Engineering under Snow and Ice.”